
All around us we find examples of turbulent, particle-laden fluids, from diseases transmitted by respiratory particles, to the dispersion of wildfire particles, volcanic ash or microplastics in the atmosphere, to droplets and ice crystals inside clouds. The physics of these examples are quite similar in many aspects, but each also has unique characteristics. Exhaled particles are released as liquid particles of varying sizes (from nanometers to millimeters) and are the main route of spread for airborne-transmitted diseases. In contrast, volcanic ash or microplastics are solid, non-spherical particles that can travel thousands of kilometers in the atmosphere and pose various kinds of risks. Clouds are probably the most complex example of all, being a mixture of aerosols, water droplets, and/or ice crystals with physics and chemistry ranging from nanometers to kilometers. In all of these examples, turbulence plays an integral role in the mechanisms involved. The physics of such particle-laden turbulent flows is extremely complex, which is why clouds and aerosols are the major source of uncertainty in weather models and climate projections, or why unexpectedly large microplastics can be found in the Arctic.
In my group, we are investigating various aspects of these problems through numerical, experimental, and field investigations. Below is a brief synopsis of our ongoing research projects.

Clouds and aerosols together with their interactions remain the largest sources of uncertainty in weather and climate models. In particular, their interactions with aerosols and their turbulent nature are the biggest challenges that remain to be understood. In our group, we have developed the Max Planck CloudKites (MPCK) and a suite of instruments to study aerosols and droplets within the atmospheric boundary layer and clouds. Our instruments bring some of the most advanced imaging techniques from the laboratory to the field, including particle image velocimetry and inline holography.
The Advanced Max Planck CloudKite (MPCK+)
The advanced CloudKite instrument box, MPCK+, consists of standard sensors and advanced imaging instruments carefully designed to maximize the overlap between the probing volumes of the different instruments. The two main imaging instruments of MPCK+ are the Particle Image Velocimetry (PIV) and the in-line Holography modules. These modules enable us to measure the size, spatial distribution, and velocity of cloud drops with unprecedented accuracy and resolution.
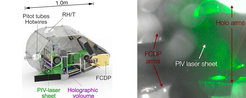
(Animation)
Airborne Particle-Tracking In-line Holography Setup: The HoloTrack
The HoloTrack instrument is the newest addition of instruments for cloud droplet measurements with the CloudKite.
By analyzing a single hologram recorded with HoloTrack, we can extract information about the cloud droplets within the 3-dimensional sample volume inside clouds.
The novel feature of HoloTrack, however, is the tracking of droplets between two holographic frames. Therefore the droplet velocity can be calculated. The uniquely short inter-frame distances of the hologram pairs are short enough to enable tracking through the combination of a short inter-frame time by timing laser pulses with the camera exposure right and the low true airspeed of the ClodKite.
HoloTrack therefore not only identifies 3D positional data, particle shapes, and sizes, but also the particle velocities within a maximum volume of 100cm^3. Cloud droplets sizing down to 6µm in diameter are resolved. The instrument is fully automated and equipped with 1D and 3D Pitot tubes, position sensors, and a Cloud Droplet Probe.
With the integration of HoloTrack, we anticipate further insight into the spatial-temporal properties of cloud microphysics, thereby deepening our understanding of clustering, spatial inhomogeneities, and mixing processes in clouds.
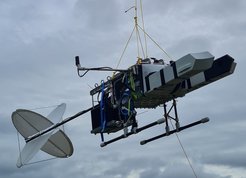
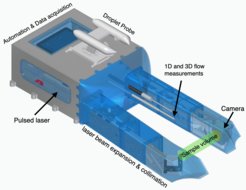
The WinDarts
The WinDarts system is an instrument box designed to enhance our understanding of atmospheric turbulence, focusing on in situ measurements within the planetary boundary layer (PBL). Each WinDarts probe is lightweight (~15 kg) and versatile, equipped with sensors that gather high-resolution 3D wind velocity data, as well as temperature, relative humidity, pressure, carbon dioxide, volatile organic compounds, and particle size distributions. All measurements are linked to time and geolocation for comprehensive analysis.
Deployed by hanging from the main tether of the CloudKite (see photo), the compact, aerodynamic WinDarts can be safely and efficiently used also in challenging weather conditions. By penetrating clouds and measuring turbulence even in regions of strong wind shear, WinDarts capture detailed insights into atmospheric dynamics. These data help elucidate the behavior of boundary layers, including their depth, stability, and interactions with the Earth's surface. Additionally, these measurements are essential for studying the transport of heat, moisture, and momentum within the PBL.
WinDarts is a robust, field-tested system that has contributed significantly to recent MPI-DS research campaigns. These include the Pallas Cloud Experiment (PaCE) in September 2022, where two first-generation WinDarts were deployed, and IMPACT (In-situ Measurement of Particles, Atmosphere, Cloud, and Turbulence) from May to June 2024. During IMPACT, up to six second-generation WinDarts were successfully launched (see photo) in scientific flights over Pallas, Finland.
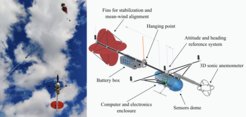

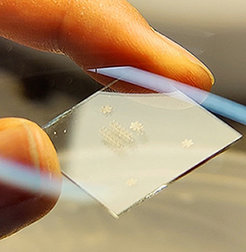
Non-spherical particles are omnipresent in the Earth's atmosphere, such as pollen, dust, volcanic ash, microplastics, and snowflakes. Although there has been a great deal of research on the dynamics of such particles, there are still significant uncertainties about their transport dynamics in the atmosphere. While the dynamics of ice crystals is central to understanding the microphysics of mixed-phase/ice clouds and their interaction with solar radiation, the transport of dust/ash/microplastics is crucial for environmental pollution control. The shape and size of these particles cover a wide range, making the description of their dynamics challenging even in quiescent flows.
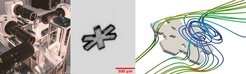
Our team has built a novel high-precision experimental setup - the Göttingen Turret - to observe the settling dynamics of non-spherical particles. We use four high-resolution cameras combined with a precision setup to track the translational and rotational dynamics of submillimeter or larger particles as they fall in quiescent air. The particles are fabricated using the NanoScribe 3D printer, which can print at submicron resolution utilizing the two-photon polymerization technique. In addition, we perform full-resolution three-dimensional simulations of free-falling particles using an in-house version of the Palabos code (https://palabos.unige.ch).
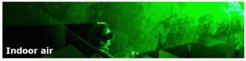
Modern people spend approximately 90% of their time indoors. This highlights the importance of indoor air quality for our well-being. It is therefore important to know which methods are best suited to improve indoor air quality and, for example, prevent the risk of infection transmission. In particular, with respect to the risk of airborne transmission of diseases, understanding the spatial and temporal evolution of aerosols indoors is critical. Differences in ventilation are critical in determining whether none, some, or all become infected. While most studies use the concept of a well-mixed room, it is clear from the header image above that the local concentration is subject to large variations.
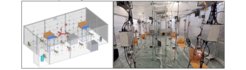
In our investigation to quantify aerosol transport in two large indoor spaces, such as German hardware stores, we found that the local particle residence time was more than an order of magnitude less than the particle residence time calculated using the nominal air exchange rate. We also designed and built a prototype classroom and equipped it with 125 optical particle counters and temperature, humidity, pressure, and trace gas sensors. In this room, we measure the local concentration of trace gases and the size distribution of particles. The key question to be answered is how inhomogeneity in aerosol concentration affects the risk of infection for people in different parts of the classroom and how we can provide safer indoor air for students in schools.
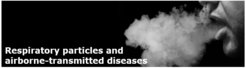
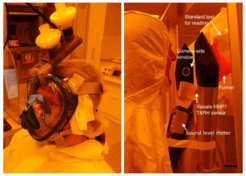
Shortly after the arrival of COVID-19 in Germany, we realized from literature searches and public discussions that both the emission of particulate matter via human exhalation and the airborne transmission of pathogens were not fully understood. Our plan was to study both phenomena in an interdisciplinary manner. To this end, we set up an aerosol and particle measurement facility in our cleanroom laboratory to study the size, concentration, and origin of human exhaled particles and their dependence on human factors. We use commercially available aerosol size spectrometers covering a range of 0.01 to 10 µm particle diameter, and a custom-built in-line holography unit to measure particles larger than 6 µm. Measurements were performed using full face masks and in an open path configuration. By examining the exhaled particle size distributions of 132 individuals ranging in age from 5 to 80 years, we found a strong correlation between age and emitted particle concentration. We also observed differences in particle size distribution and number concentration within a sub-cohort of 31 wind instrument players.
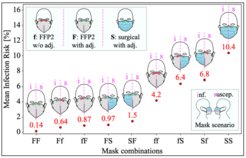
By combining our measurements of exhaled respiratory particles from 132 subjects, measuring masks of different fit/type in seven subjects, and using improved physical/mathematical models, we assessed the risk of airborne transmission of COVID-19 during breathing and speaking activities. Even with an upper bound for infection, masks were found to be highly effective in preventing airborne transmission of COVID-19. This plots provided a key piece of evidence for the efficacy of face masks to policymakers, influential individuals, and the general public during the Covid 19 pandemic, as indicated by the study's Altmetric score. Here "i" represents the type of mask worn by contagious individuals and "s" represents the mask worn by susceptible individuals. "w/o adj." means that the mask is worn without fitting to the face, and "with adj." means that the mask was fitted to fit the wearer's face as well as possible (manual fitting without using a fit test).